7. Metabolism#
In the previous chapters, you’ve learned about how enzyme catalysis accelerates biochemical reactions, how external signals control enzyme activity, and how thermodynamics determines the feasibility of reaction outcomes. But how are these tools used to build a living cell from simple nutrients? Which enzymes in a metabolic pathway tend to be regulated by allosteric binding sites? Which enzymatic steps use energy carriers to increase reaction favorability, and why? What are the benefits to the cell?
In this chapter, we will start to put everything together in cellular context of biochemical reactions and biochemical regulation. You will start to see how metabolic pathways harness the properties of enzymes to ensure cell growth and maintenance in a wide variety of conditions. By the end of the chapter, you will begin to see how an organism can make the right amount of building blocks from nutrients scavenged from the environment, and do so with incredible efficiency.
7.1. Basic concepts of metabolism#
7.1.1. Metabolism is organized into metabolic pathways that convert precursors into products#
The chemical products generated by the breakdown of nutrients require multiple sequential chemical transformations to be converted into a chemical product such as an amino acid. These series of transformations are known as metabolic pathways. This term “metabolic pathway” may also refer to the group of enzymes that catalyze the chemical transformations. Fig. 7.1 depicts the proline biosynthesis pathway, which converts the amino acid glutamate (the precursor) into the amino acid proline (the product).
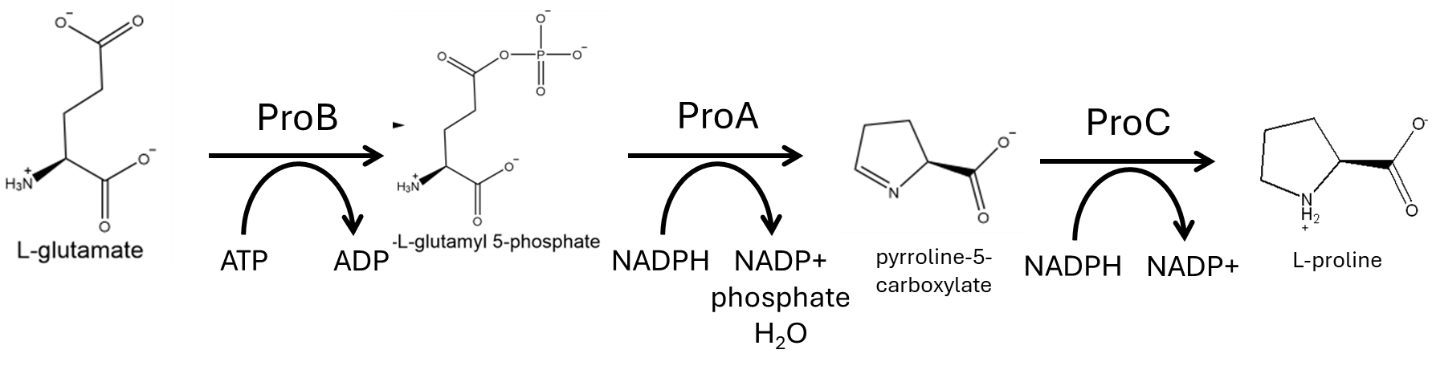
Fig. 7.1 The proline biosynthesis pathway.#
Each transformation of a metabolic pathway is usually catalyzed by an enzyme (some very rare reactions occur spontaneously without any assistance from a catalyst). For instance, the transformations that convert glutamate into proline are catalyzed by three enzymes that work in sequence (ProB, ProA, and ProC). The products of the enzymes within the pathway preceding the final product are referred to as intermediates. Sometimes these intermediates can also serve as precursors for other pathways. When this happens, the pathway is said to be a branched pathway, as the pathway “branches” from one compound into two pathways with different products. However, many metabolic intermediates have no purpose beyond the metabolic pathway.
7.1.2. Catabolic and anabolic pathways#
Metabolism is the conversion of nutrients (e.g. glucose, ammonium, and phosphate) into the building blocks of living cells (amino acids, nucleotides, fatty acids). Metabolism is conventionally divided into catabolism and anabolism.
Catabolism represents the first half of metabolism: the conversion of nutrients into metabolites. Catabolic pathways exist for specific nutrient sources: glucose is converted into pyruvate (and other useful intermediates) by the glycolytic pathway; fatty acids are catabolized into acetyl-CoA (and NADH) by the fatty acid degradation pathway (also known as beta-oxidation); amino acids may be converted to basic metabolites such as acetyl-CoA by specific amino acid degradation pathways; carbon dioxide is catabolized by carbon fixation. As you can imagine, specific pathways with distinct enzymes are needed for each potential nutrient because enzyme active sites are highly selective for substrates. In addition to generating basic metabolic building blocks such as acetyl-CoA, pyruvate, 2-ketoglutarate, and glycerone-3-phosphate (to name a few), catabolic pathways may also generate compounds involved in high-energy group transfers (e.g. ATP, NADH, NADPH, and acetyl-CoA). Of course, not all organisms possess all catabolic pathways. Many bacteria specialize into ecological niches based upon the nutrients they are able to consume via catabolism. For instance, bacteria that are able to fix atmospheric nitrogen will have an advantage in a nitrogen-poor environment over bacteria that rely upon fixed nitrogen sources.
Anabolism represents the second half of metabolism: the conversion of metabolites into the building blocks of living cells. Specific anabolic pathways exist for all required building blocks (all 20 amino acids, etc.), but not all organisms possess all anabolic pathways. Organisms that lack specific anabolic pathways must acquire the building block as a nutrient – this is why mammals must be sure to acquire 9 essential amino acids from their diet, and why some bacteria must be supplied with fatty acids to build membranes as they lack fatty acid synthesis pathways. E. coli possesses all pathways needed to grow using glucose, ammonium, and phosphate as sole nutrient sources. Mutants of organisms that lack an enzyme that participates in an anabolic pathway for an amino acid (or another building block) are referred to as auxotrophs. For instance, a tyrosine auxotroph would need to obtain tyrosine from the environment in order to grow. The inability to synthesize certain essential nutrients also constrains the ecological niches accessible to different organisms.
Anabolism (and when needed, direct nutrient uptake from the environment) supplies the processes that generate biomass (DNA, RNA, proteins, membranes). These processes include DNA replication, RNA transcription, and protein translation. When these processes lack one of the building blocks required, the process stops and further cell growth is impossible. Many organisms have mechanisms to ensure that the different biomass-generating processes are synchronized – for instance, in many bacteria, inhibition of protein translation due to lack of an amino acid triggers synthesis of a signal molecule that arrests transcription by RNA polymerase.
7.1.3. The steady-state concentrations of metabolites are determined by the ratio of the rates of metabolite synthesis divided by the rates of metabolite consumption#
A cell growing in stable conditions is said to be at steady-state. In this state, despite the fact that the cell continues to grow and divide and cell concentrations are increasing in the environment, the concentrations of metabolites and proteins inside the cell remain relatively stable.
Considered from the standpoint of a metabolic pathway, the steady-state condition implies that the fluxes (the number of substrate molecules being converted into products, minus the number of product molecules being converted back into substrates) are equal for each individual chemical reaction in the pathway. This is because unequal fluxes would result in an accumulation or depletion of one of the intermediates. Since this would change the concentration of the intermediate, this situation is by definition not in steady-state. Note that the concentrations of the enzymes or the substrates in the pathway may not be equal at steady-state.
7.2. Regulatory principles of metabolic pathways: biosynthetic pathways of anabolism as a general example#
Recall the Michaelis-Menten equation relating reaction rate to substrate and enzyme concentrations: for a single reaction at the steady-state, the rate of synthesis of product is equal to the rate of consumption for substrates. When a pathway exists at the steady state, all substrates and all products are consumed and synthesized at equal rate, respectively:
If Enzyme B catalyzes a single-substrate reaction, then at the steady state, rate of synthesis of Intermediate 2 will be:
And, at the steady state, the reaction rates through each enzymes must be equal:
Again, this does not imply that concentrations and of enzymes and intermediates of the pathway are equal!
Metabolic pathways tend to have specific regulatory interactions that give rise to several properties:
Biosynthesis pathways do not synthesize significantly more product compound than is needed for the cell. Catabolic pathways are generally not expressed unless their specific substrate is present in the environment.
The rate at which the products of metabolic pathways are synthesized may be relatively insensitive to variation in precursor concentration (as long as some amount of substrate is available, of course)
Metabolic pathways sustain stable steady-state concentration of products
Pathways supply product at a high rate without requiring high concentrations of substrates or products.
The regulation of metabolic pathways contributes to the versatility, robustness, and adaptability of metabolism, and in turn, enables cells to grow in a wide variety of conditions and with a wide variety of nutrients.
7.2.1. Metabolic pathways self-regulate via negative feedback in response to cellular demand for product#
The growth of all cellular life forms requires the same set of building blocks: amino acids for proteins, nucleotides for DNA and RNA, and lipids for membranes. These building blocks may be obtained directly from the environment when available. However, many organisms are able to grow in the absence of these building blocks because they possess genes encoding metabolic pathways that assemble the building blocks from basic metabolites. For instance, E. coli produces all the building blocks it requires from glucose, ammonium, and phosphate (so-called “minimal medium”). However, if you feed amino acids to E. coli, it no longer has any need to produce the amino acids itself. Many amino acid biosynthesis pathways will then be repressed, and the cell will stop expressing the genes encoding the biosynthesis pathway enzymes. This is economical because it is more efficient for E. coli to take up environmental amino acids than to commit metabolic intermediates and energy towards producing the enzymes required to synthesize the amino acids itself. Specifically, E. coli will divert the resources that would be used to express amino acid biosynthesis pathways towards production of additional ribosomes. With more ribosomes, the cell will be able to translate proteins at a higher rate. Because cell growth is generally determined by the rate of protein translation, E. coli given medium containing amino acids will grow faster than E. coli given minimal medium alone. Of course, when environmental amino acids are depleted, E. coli will have to start producing amino acids again for protein translation, and the genes encoding the biosynthetic pathway enzymes are expressed.
This example illustrates how cells turn metabolic capabilities on and off depending on need. Gene expression can be induced (switched on) by specific conditions. This phenomenon was first described by Jacques Monod, who won the Nobel Prize in 1965 for his work.
How does this happen? How does enzyme expression change metabolic capabilities of a cell? In an environment lacking amino acids, cells will need to express the enzymes required to convert basic metabolites into amino acids. First, remember that the rate of product formation \(V_B\) catalyzed by Enzyme B in the example above can be described by the Michaelis-Menten equation:
\(V_B\) is a function of substrate concentration, but is also a function of total enzyme concentration, \([E_T]\). An E. coli cell starving of an amino acid will simply induce the genes encoding enzymes that when collectively expressed will catalyze the series of reactions that produce the amino acid. Gene induction increases \([E_T]\), thus increasing the product formation rate (assuming enough substrate is present, of course).
How do cells know when to express amino acid biosynthesis enzymes? This is achieved through transcriptional regulation. Remember that proteins (such as enzymes of metabolic pathways) are translated from messenger RNA by ribosomes. Messenger RNA is produced by RNA polymerase, which copies DNA sequences into RNA. Transcriptional regulation is the control of mRNA synthesis from specific genes, and thus determines the protein content of a cell.
For many bacteria such as E. coli, when an amino acid is available in the environment, the genes encoding the biosynthetic enzymes for that amino acid are transcriptionally repressed. They are expressed at very low levels (but usually not zero levels) relative to when the amino acid is absent from the environment. How does the cell know to repress transcription when amino acids are available? This is achieved via transcriptional regulators, which are usually proteins. These regulators detect (either directly or indirectly) a particular environmental variable (such as the presence of an amino acid) and either repress or activate transcription from the specific genes controlled by the regulator (such as genes encoding amino acid biosynthetic operons).
One example is the E. coli transcriptional regulatory protein ArgR, which regulates genes encoding enzymes that participate in the arginine biosynthesis pathway (Fig. 7.2). ArgR inhibits expression of arginine biosynthesis enzymes by binding to the DNA region upstream of the coding region, which prevents RNA polymerase from transcribing the genes into mRNA. Without mRNA, the enzymes are not expressed, and the cell does not produce arginine.
How does ArgR determine when to allow transcription of arginine biosynthesis genes? ArgR also binds arginine. The ArgR-arginine complex is able to repress transcription from the promoters that ArgR recognized. In the absence of arginine, ArgR is no longer able to repress transcription from the ArgR-regulated promoters. This allows RNA polymerase to transcribe the arginine biosynthesis genes and express the arginine biosynthesis pathway, which will supply the cell with arginine.
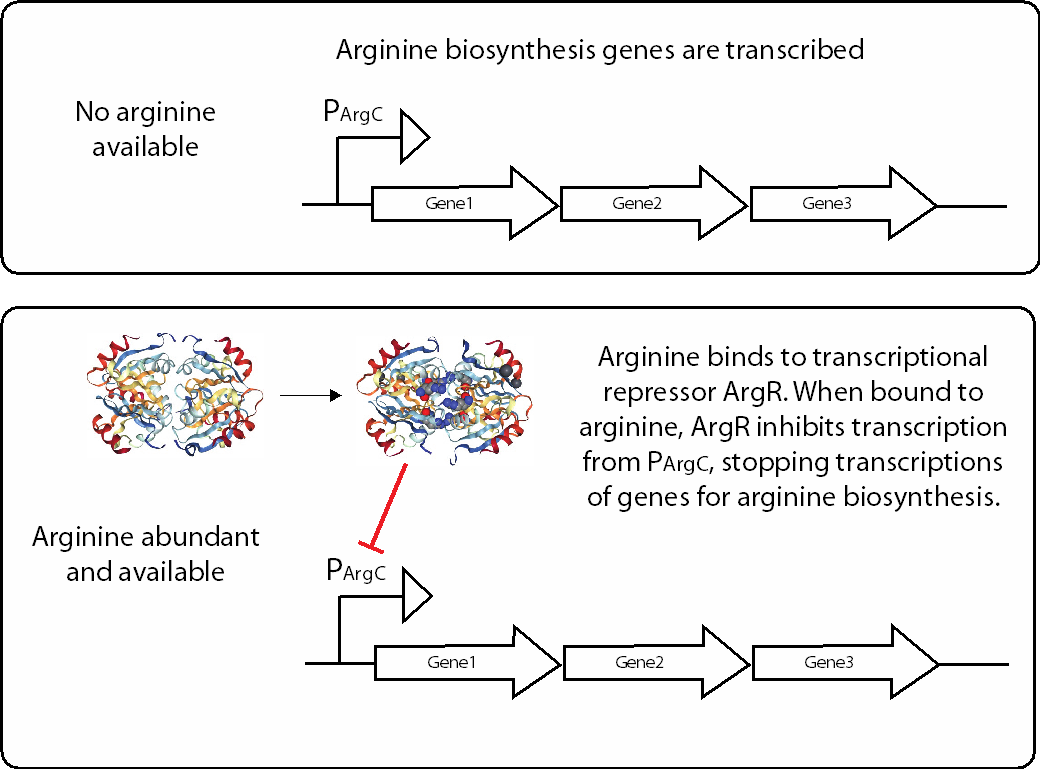
Fig. 7.2 Cells express metabolically relevant enzymes only when necessary. Amino acid arginine production is shown as example. Structure of ArgR adapted from PDB 1AXX [1].#
This mechanism is an example of transcriptional regulation via negative feedback, where the product of a pathway (in this case, arginine) inhibits expression of pathway enzymes. A negative feedback mechanism ensures that enzymes of a metabolic pathway are present only when there is a need for the product of the pathway. This form of regulation controls many metabolic capabilities that are only necessary for the cell under specific conditions: in this case, the presence or absence of arginine.
Other capabilities controlled by transcriptional regulation include catabolic pathways (conversion of external nutrients to central metabolites) such as the catabolism of the sugar lactose. Catabolic genes for most nutrients (but not all) are also expressed only when the nutrient is present in the environment. Like arginine biosynthesis, expression of catabolic genes can be induced by the presence of the specific nutrient in the environment; however, the presence of more favorable nutrients represses expression of “less-favorable” nutrients. For instance, E. coli will not use lactose in the environment if there is also sufficient glucose. This response (diauxic growth) is not mediated by negative feedback, but rather a slightly more complex regulatory network.
While many metabolic pathways are under inducible transcriptional control – which determines metabolic activity by adjusting the abundance of specific enzymes (\([E_T]\)) – other pathways are not induced by specific conditions, but are instead expressed at more or less the same level in all growth conditions. In this case, \([E_T]\) for enzymes within these pathways would remain relatively constant. These are pathways that produce metabolites that are be required in all situations – for instance, the production of metabolites that cannot be acquired from the external environment. This type of expression is known as constitutive expression. You might wonder how these pathways are regulated, since the requirement for their metabolic products will also vary over time (fast-growing cells may need more of the building block). If you remember from Chapter 4 how enzymes may be activated and deactivated by small molecules such as UTP and CTP, perhaps you can guess how these pathways can be regulated. This will be discussed further below.
7.2.2. The first (or an early) step in metabolic pathways is thermodynamically favored due to high group transfer potential#
A recurring motif of metabolic pathways is the use of high transfer potential reactions in the first reaction of the pathway (or at an early step). Specifically, the first reaction is often coupled with phosphate transfer from ATP or acetyl transfer from acetyl-CoA, which are strongly exothermic reactions. Coupling such transfers to a reaction renders the reaction effectively irreversible. There are of course examples for which the first step of the metabolic pathway is thermodynamically unfavourable without the coupling to phosphate or acetyl transfer; in those cases the need for a strong potential transfer to overcome a positive \(\Delta G\) is easier to understand (as described in Chapter 5). But this is not necessarily the case for every pathway. So why consume a “high-energy compound” in the first step of a metabolic pathway?
One advantage of making the first step of metabolic pathways strongly forward-driven is that this will make the reaction less dependent upon substrate concentrations. To understand why this is an advantage, imagine the alternative case in which the first step of the pathway is highly reversible (\(\Delta G'^{\circ}\) ~ 0 kJ/mol) (also see Figure 7.3). In this case, driving the reaction forward to generate a high abundance of the first metabolic intermediate will require a correspondingly-high concentration of metabolic precursor, which may not always be possible in all conditions. The highest concentration of Intermediate 1 achievable for such reactions would be equal to the concentration of the precursor. Furthermore, the reaction will be less efficient since the reverse reaction will occur frequently as the concentration of metabolic intermediate 1 increases towards equilibrium. However, if the reaction is coupled with (for example) phosphate transfer from ATP, then a high concentration of substrate is no longer required to make the reaction thermodynamically favorable (of course, some precursor must be available, and the high-energy compound must also be available). Furthermore, the frequency of the reverse reaction (intermediate back to precursor) is considerably reduced because it becomes highly thermodynamically unfavorable. This ensures that the enzymes are effectively catalyzing only the forward reaction.
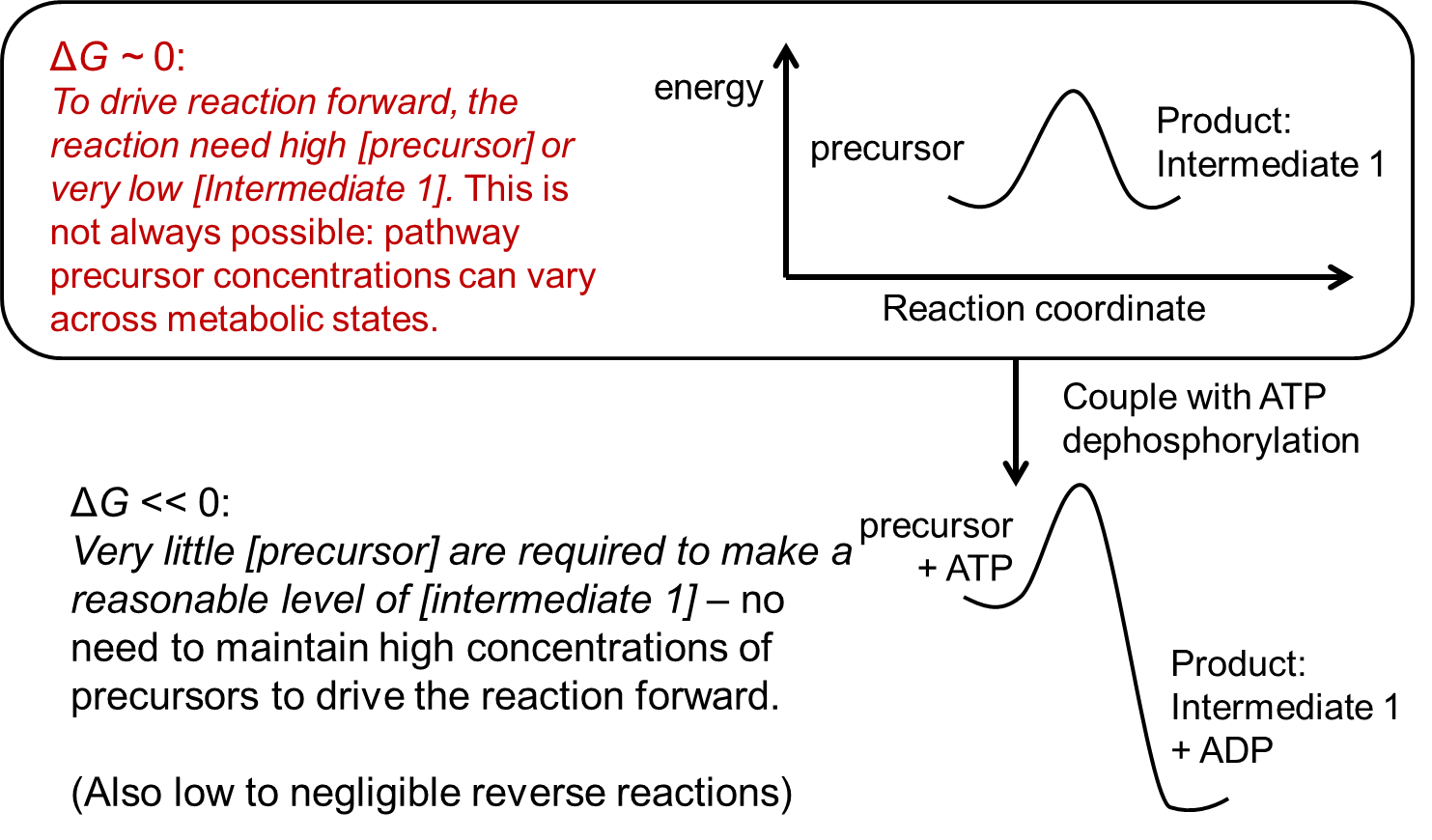
Fig. 7.3 Advantage from coupling reactions to exothermic group transfers.#
7.2.3. Metabolic regulation ensures that product concentrations remain stable#
As described above, many (but not all) pathways are expressed only in conditions in which they are needed. This reflects a form of transcriptional control. However, many pathways are constitutively expressed. Furthermore, transcriptional control is not very exact, and it is also slow to respond: expressing genes in bacteria (from inducing transcription to accumulating expressed protein) requires several minutes; in eukaryotes, this process takes hours or days. Since metabolic conditions can change rapidly, how does metabolism ensure that the products of biosynthetic pathways are never too abundant?
As it turns out, biosynthetic pathways are controlled at the post-translational level by product inhibition. Specifically, the product of the metabolic pathway (amino acid, nucleic acid, etc.) inhibits the activity of an enzymatic reaction early within the pathway. This regulation – another form of negative feedback – is often achieved by allosteric inhibition, which you encountered in Chapter 4. This form of regulation is called post-translational regulation (i.e. regulation of enzymes after they are translated by the ribosome) and is an added layer of control in addition to transcriptional regulation. Recall the discussion on the enzyme ATCase in Chapter 4 and its role in balancing concentrations of pyrimidine triphosphates (UTP and CTP) with purine triphosphates (ATP and GTP).
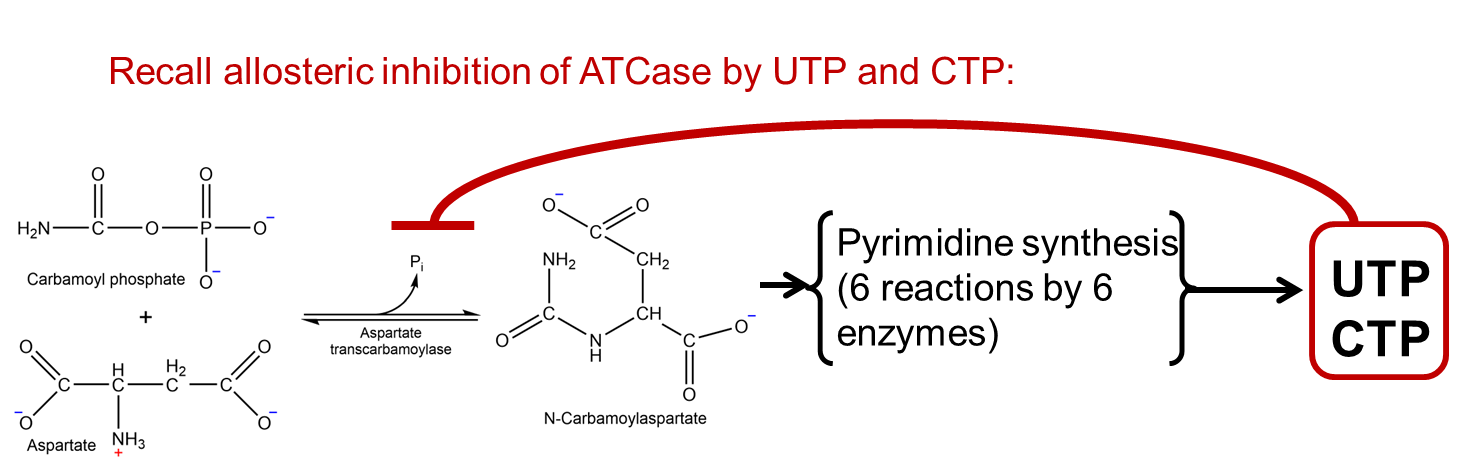
Fig. 7.4 .#
What are the benefits of allowing the metabolic product to inhibit the first enzyme of the pathway? There are two answers to this question. The first answer is clear: once the product accumulates to some concentration, there is less need to continue to synthesize the product. Thus, the product inhibits the first enzyme of the pathway, which prevents unnecessary production, thus saving precursor metabolites, any cofactors needed for enzymatic steps in the pathway, and accumulation of the product to concentrations that may be toxic to the cell.
The second answer is however less clear. When the concentration of the product of the pathway has reached its target concentration, subsequent allosteric inhibition of the first enzyme (and decreasing flux through the pathway) will effectively place the pathway in a less-activated state: so the pathway is operating at (for instance) only 50% of its synthesis capacity. This may sound like an inefficient strategy: why keep half of your enzymes inhibited instead of expressing fewer enzymes? However, this seems to be the situation in may biosynthetic pathways for which metabolic products have been quantified. The strategy does have one considerable advantage: if concentrations of the product decrease for any reason (e.g. stress or increased requirement for the product), inhibition of the first enzyme will be immediately released, its activity will increase, and metabolic flux will flow though the pathway once again to increase the product back to its initial levels. Thus, the product concentration will tend to stay at a fixed value. For essential cellular processes such as protein translation, this helps to ensure that amino acids are maintained at a specific concentration.
Of course, if re-activation of the pathway by release of allosteric inhibition is insufficient to supply the cell with the metabolic product, the cell may then need to increase the total abundance of the pathway enzymes. This response is mediated via transcriptional regulation. These two forms of negative feedback control: post-translational regulation (due to allosteric inhibition of the first pathway enzyme by the pathway product) and transcriptional regulation (inhibiting expression of the pathway enzymes when the product is available from the external environment) both serve similar purposes. However, post-translational regulation acts far quicker than transcriptional regulation.
7.3. An in-depth view of central carbon metabolism: the glycolysis pathway#
Glycolysis is one of the most central metabolic pathways. The pathway is far more useful than its name would imply: far more than the processing of conversion of one glucose molecule into two pyruvate, ATP, and NADH molecules, reactions within the glycolysis pathway also generates metabolic precursors for amino acids and phospholipid head groups. In fact, only the first two reactions are truly glucose-specific: other sugars such as fructose are also processed via the pathway. Furthermore, even in the absence of sugars, many of the reactions within the pathway serve the reverse pathway (gluconeogenesis) to generate the essential metabolite glucose-1-phosphate. The reactions are thus referred to as “glycolysis” more as a historical accident than anything else.
In the context of a human cell, the ATP generated from glycolysis is insufficient for the needs of most cells. Thus, much of the pyruvate generated is fed into the TCA cycle and further oxidized in the mitochondria via electron transport. This is a process called cellular respiration and generates many ATP molecules, as you will learn about later. However, the glycolysis pathway can be relied upon exclusively for the generation of ATP in certain cases, but especially in the absence of oxygen. E. coli will use glycolysis as the primary source of ATP, even in the presence of oxygen, and will entirely skip using cellular respiration to generate ATP.
Interestingly, eukaryotic cells that grow and divide rapidly also rely more upon glycolysis as an ATP source than cellular respiration via the electron transport chain. Two examples are proliferating white blood cells (needed to rapidly respond to infections) and also rapidly-proliferating cancer cells. The reason for why glycolysis is used instead of cellular respiration for ATP synthesis by cells as diverse as E. coli, baker’s yeast, activated white blood cells, and tumor cells in spite of the apparent wastage of carbon (all that ATP that is not being obtained) is fascinating but is beyond the scope of this introduction!
In this section, we will explore glycolysis and use it to illustrate several of the principles you have already learned about in this course:
How does architecture of glycolysis adhere to the principle of efficiency in metabolism?
Why do the initial steps occur in the order that they do?
Where does the ATP come from?
Where does the NADH come from?
What happens if we run out of NAD+?
Which steps are energetically “disfavored”?
Which steps are driven by Le Chatelier’s principle?
Often, students think of glycolysis are taught to memorize the individual steps of the pathway instead of the reasons for why the pathway reactions occur in the order that they do. Like all pathways, glycolysis must obey the laws of thermodynamics as well as laws of chemical reactions. Given these constraints, glycolysis has evolved to be highly efficient in its strategy as we will see.
7.3.1. The overall structure of glycolysis is divided into upper and lower parts#
Glycolysis is often broken down into two parts: upper glycolysis, and lower glycolysis, each with different end points:
Upper glycolysis: a 6-carbon molecule (glucose) is phosphorylated twice (costing two ATP molecules) and then broken down into two 3-carbon molecules (glyceraldehyde-3-phosphate and dihydroxyacetone phosphate), which are readily intercoverted by an isomerization reaction.
Lower glycolysis: Glyceraldehyde-3-phosphate (G3P) is further processed via a series of oxidation, phosphorylation and phosphate transfer steps to yield pyruvate, an NADH molecule, and two ATP molecules.
Since one glucose yields two G3P molecules that each yield one NADH and two ATP, the total benefit is two pyruvate, two NADH, and two ATP molecules per glucose (two ATP spent in upper glycolysis; four ATP gained total in lower glycolysis).
7.3.2. The goal of upper glycolysis: generate two identical molecules from an asymmetric precursor#
Looking at how the upper and lower parts of glycolysis fit together we can see an advantage of its current arrangement. Upper glycolysis generates two identical molecules (G3P) that can both be subsequently processed by the same pathway (lower glycolysis). This is advantageous over a hypothetical alternative pathway that splits the 6-carbon glucose molecule into a 4-carbon and a 2-carbon intermediate. A pathway that handled glucose this way would then need to process the 4- and 2-carbon intermediates via different pathways, which is less efficient than using lower glycolysis to process two copies of the same molecule (G3P). This gain in efficiency is useful to help understand why the reactions in upper glycolysis happen the way they do.
Now let’s look at the reactions in more detail. Upper glycolysis consists of a phosphate transfer (ATP to glycose) to generate glucose-6-phosphate (G6P). G6P is then isomerized to fructose-6-phosphate, which is subsequently phosphorylated again by a phosphate transfer from ATP to fructose-1,6-bisphosphate (F1,6BP). In the key step of the pathway, F1,6BP is broken into two three-carbon molecules: G3P, and DHAP. DHAP is isomerized to G3P.
Why do the reactions occur in the order that they do? In particular, what is the purpose of that isomerization step (G6P to F6P)? The short answer is that this isomerization step is required to eventually generate two molecules of G3P. Without this isomerization, two non-identical molecules (4- and 2-carbons) would be generated. Why is this?
The answer is rooted in organic chemistry: carbon-carbon bonds are extremely stable and in general difficult to break. However, carbon-carbon bonds can be weakened if the carbons forming these bonds share electrons with nearby electronegative atoms such as oxygen. In the case of glucose, carbon-carbon bonds that are “beta” to carbonyl carbons (those with a double bond with oxygen) are weaker than ordinary carbon-carbon bonds by a factor of 1033.
Looking at the structure of glucose, there appear to be no carbons with a double bond to any oxygen. However, one of the carbons has the oxidation state of a carbonyl carbon: carbon 1, which has a bond with the oxygen atom within the glucose ring and an additional oxygen. As it turns out, this functional group (called a hemiacetal) exists in equilibrium with the carbonyl form and as such they interconvert in aqueous solution.
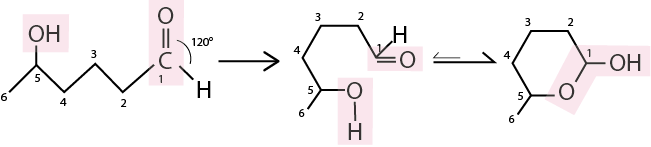
Fig. 7.5 A cyclic hemiacetal exist in equilibrium between linear (left) and cyclic (right) form, with the cyclic form heavily prevalent at equilibrium.#
A linear graphical representation of the sugars helps visualize how the glucose-to-fructose isomerization changes the position of the weakest bond, and why the isomerization reaction (second reaction within the glycolysis pathway) makes upper glycolysis efficient:
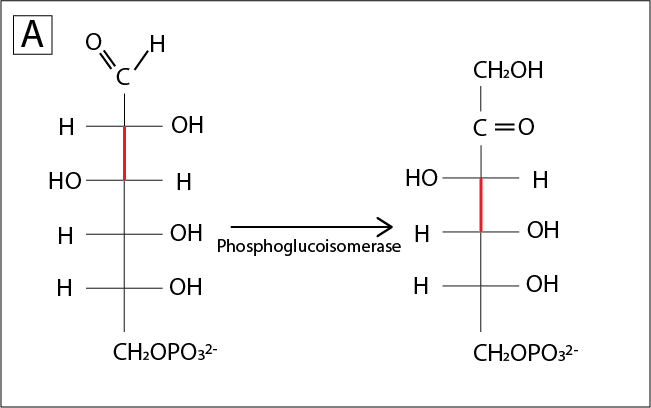
Fig. 7.6 ..#
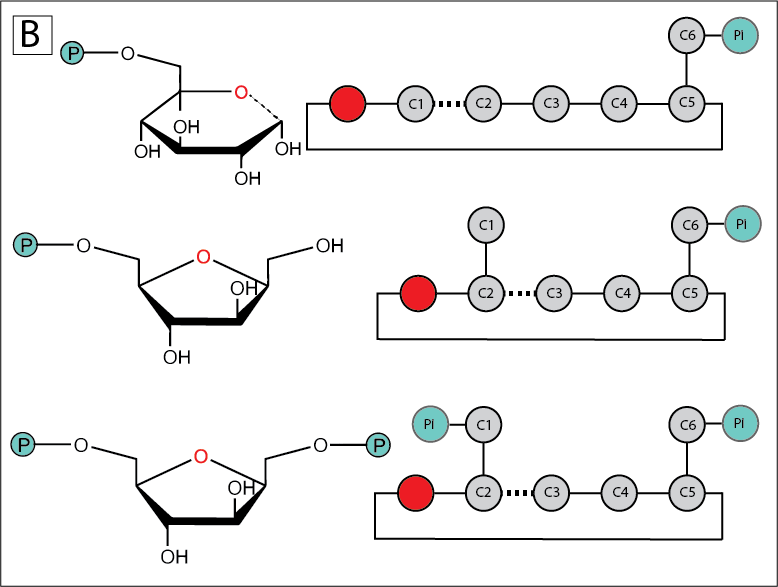
Fig. 7.7 (A). Isomerization moves the position of the weakest C-C bond, the bond in beta position to the hemiacetal, one carbon down the chain. (B) The catalytic isomerization of glucose to fructose promotes the following generation of two 3-carbon chains in place of one 2-carbon and one 4-carbon chains.#
Following the isomerization, the enzyme phosphofructokinase catalyzes the transfer of a phosphate group to fructose 6 phosphate, to generate fructose 1,6 biphosphate. Enzyme aldolase cleaves fructose 1,6 biphosphate into DHAP and G3P:
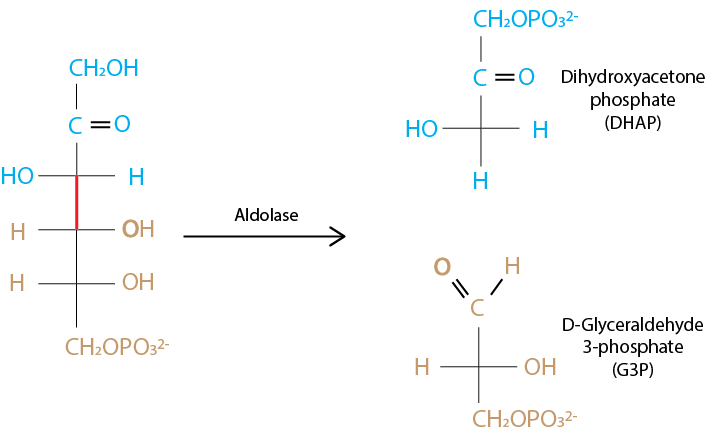
Fig. 7.8 Cleavage of sugar 6-carbon chain to two triose phosphates is catalyzed by enzyme aldolase.#
The next step is to isomerize DHAP into G3P, to make two identical 3-carbon molecules. This reversible isomerization is extremely rapid and allows the cell to funnel two identical molecules of G3P into one pathway instead of evolving multiple enzymes that need DHAP as a precursor.
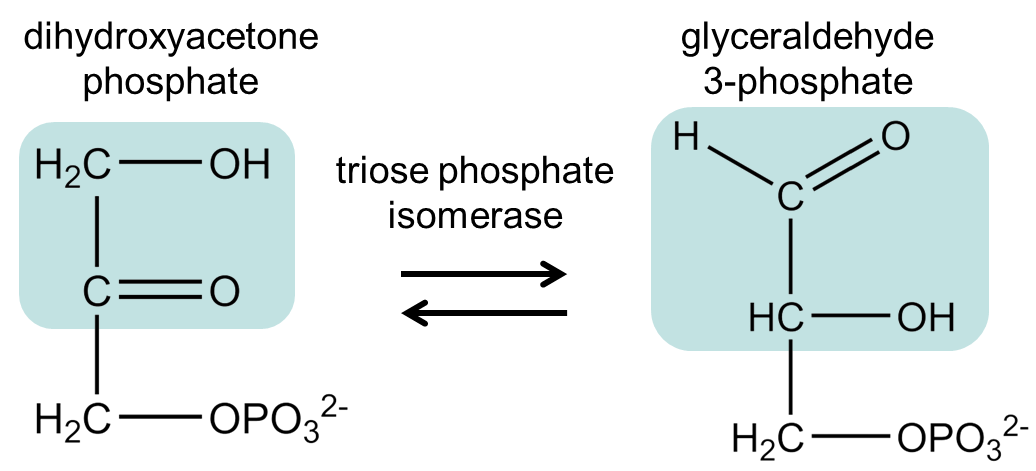
Fig. 7.9 .#
7.3.3. Lower glycolysis generates ATP and NADH#
Now we have invested one glucose molecule and two ATP to generate two molecules of G3P that will be eventually converted into pyruvate. Along the way, we will also generate two molecules of ATP and one molecule of NADH per G3P. The next section will look the remaining steps of glycolysis, focusing on the following questions:
Where does the ATP come from?
Where does the NADH come from?
What happens if we run out of NAD+?
Which steps are (seemingly) energetically disfavored?
Which steps are driven by Le Chatelier’s principle?
G3P oxidation generates a compound with high phosphate transfer potential. The first reaction of lower glycolysis, catalyzed by G3P dehydrogenases, converts G3P to 1,3-bisphosphoglycerate. In doing so, the enzyme oxidizes G3P using NAD+ and adds a phosphate group to the new carboxylic acid. This is an oxidation reaction because the aldehyde group in G3P has been oxidized to the equivalent of a carboxylate group (three bonds to oxygen) with one of the oxygens part of a phosphate group (a carbonyl group bound to a phosphate in this way is called a mixed anhydride and has a high phosphate transfer potential). Since G3P was oxidized, this means that NAD+ must be reduced to NADH. This yields our first reducing equivalent, which may come in handy later.
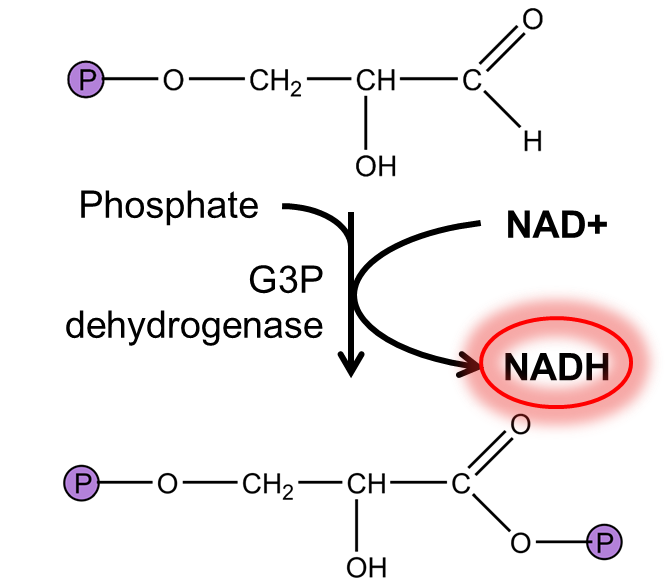
Fig. 7.10 .#
This reaction is not favourable in standard conditions (ΔGʹ0= +6.3 kJ/mol) and is thus a useful example to demonstrate how cells must maintain reactants at concentrations that allow reactions to proceed. The NADH/NAD+ ratio, which in glucose-utilizing E. coli is maintained at very low levels (~0.01), is particularly important here. This means that for glycolysis to proceed, cells must ensure that NADH > NAD+. For multicellular eukaryotes, this is accomplished in most conditions by oxidative phosphorylation (breathing) which re-oxidizes NADH to regenerate NAD+ by transferring electrons to oxygen. However, when not enough oxygen is available, some tissues can transfer electrons to pyruvate, as will be discussed below.
Our first ATP: phosphoglycerate kinase transfers phosphate from 1,3-bisphosphoglycerate to ADP. The mixed anhydride in 1,3-bisphosphoglycerate has a higher phosphate transfer than ATP and as such is able to generate ATP from ADP. This phosphate transfer is catalyzed by phosphoglycerate kinase, and has an extremely favourable standard free energy (\(\Delta G'^{\circ}\) = -18.5 kJ/mol). (Given the standard free energy of ATP hydrolysis, can you calculate the standard free energy of 1,3-bisphosphoglycerate hydrolysis?) Note that as this reaction involves a phosphate transfer, phosphoglycerate kinase uses the induced-fit mechanism (just like hexokinase) to ensure that phosphate does not transfer from 1,3-bisphosphoglycerate to water.
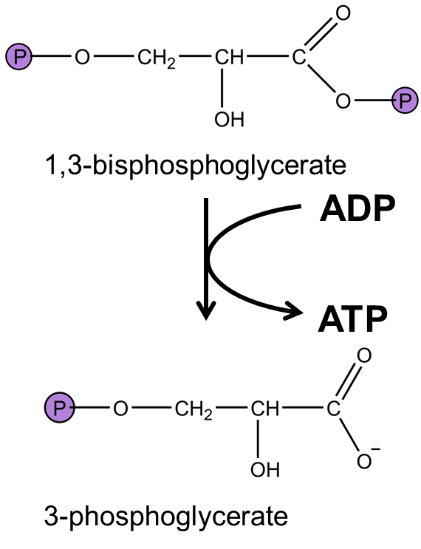
Fig. 7.11 .#
An isomerization and a dehydration sets us up for a final phosphate transfer to generate ATP. Phosphoglycerate mutase and enolase rearrange 3-phosphoglycerate into phosphoenolpyruvate (PEP), a molecule with tremendously high phosphate transfer. Pyruvate kinase catalyzes the final phosphate transfer from PEP to ADP, a very favorable reaction (\(\Delta G'^{\circ}\) = -31.4 kJ/mol – can you calculate the free energy of PEP hydrolysis from this value?).
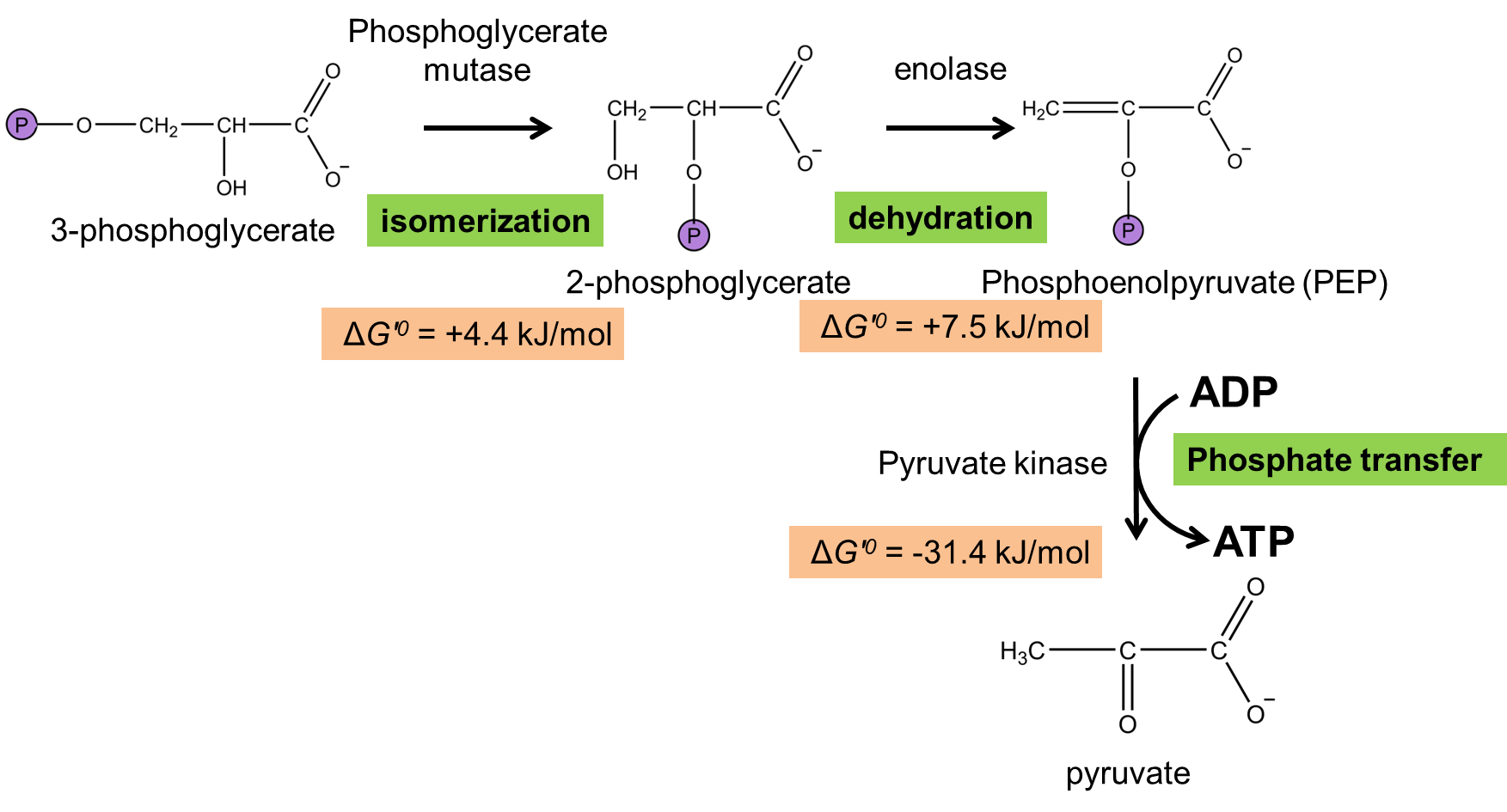
Fig. 7.12 .#
By generating molecules with extremely high phosphate transfer (1,3-BPG and PEP), glycolysis is able to make it thermodynamically possible to generate a total of 4 ATP from an initial investment of 2 ATP, 2 NAD+, 2 phosphate ions, and 1 glucose molecule. Glycolysis also generates two pyruvate molecules. Pyruvate can be converted to the amino acid alanine, or into acetyl-CoA for assembly into fatty acids or delivery into the TCA cycle for conversion into amino acids or for oxidative phosphorylation.
NEED TO KNOW
From an investment of 1 glucose, 2 ATP, and 2 NAD+, the total output of glycolysis accounts to 4 ATP, 2 NADH, and 2 pyruvate.
Therefore, the net energetic benefit is 2 ATP and 2 NADH per molecule of glucose.
7.3.4. Fermentation: how cells regenerate NAD+ to keep glycolysis moving when electron acceptors are absent#
In order to glycolysis to proceed, the cell must maintain a high NAD+/NADH ratio. This requires electron acceptors to convert NADH back into NAD+. Physiological electron acceptors include, for example, gaseous oxygen (O2) which is reduced to water thanks to the electrons transferred from NADH during oxidative phosphorylation. Transfer of electrons from NADH into the electron transport chain regenerates NAD+, which maintains the high NAD+/NADH ratio required for glycolysis. You will learn more about this in a subsequent chapter.
In the absence of other electron acceptors, the cell will use products of glycolysis as electron acceptors. Pyruvate, the final product of glycolysis, can be reduced to lactate by receiving a hydride ion (H-) from NADH, which regenerates NAD+. This generation of lactate happens in our muscles during periods of high exertion when the tissue cannot supply oxygen at a rate sufficient to oxidize NADH to regenerate NAD+.
Yeast, eukaryotic unicellular microorganisms, use a slightly different fermentation pathway: pyruvate is first decarboxylated to acetaldehyde (releasing carbon dioxide). Acetaldehyde is subsequently used to regenerate NAD+ from NADH by reduction to ethanol.
Note that fermentation does not yield additional ATP, but recycles the NAD+ consumed by glycolysis, therefore indirectly allowing glycolysis to continue by support the maintenance of a high intracellular NAD+/NADH ratio required to sustain glycolysis.
Fabio Arnesano, Lucia Banci, Ivano Bertini, and Isabella C. Felli. The solution structure of oxidized rat microsomal cytochrome b5,. Biochemistry, 37(1):173–184, 1998. doi:10.1021/bi971896w.