1. Biomolecules#
1.1. Introduction#
In this chapter we will introduce some of the important classes of molecules in biochemistry. We will start by talking about carbohydrates (sugars). Then we will discuss the main types of lipids (fats) and their functions. Finally, we will discuss the amino acids and their properties.
1.2. Sugars#
One of the most important classes of biomolecules are the carbohydrates, otherwise known as sugars. Carbohydrates are defined as carbon-based molecules rich in hydroxyl (-OH) groups (i.e. (CH2O)n). These compounds are energy dense, though less than lipids, and because of this carbohydrates are an important source of energy for most organisms. Sugars, or saccharides, come in many different varieties and arrangements. You can have a single or monomeric sugar, a monosaccharide, or multiple sugars attached to one another, a di- or polysaccharide. Furthermore, sugars can be used to modify other classes of biomolecules. This third, special class of sugars are the glycoproteins and glycolipids, where the sugars are linked to either proteins or lipids, respectively.
1.2.1. Monosaccharides#
There are many types of monosaccharides, most of them composed of anywhere between 3 and 6 carbon atoms, though there exists also a 7 carbon sugar, heptulose. The most well-known are molecules like glucose, fructose and (deoxy-)ribose. Six carbon atoms, like in glucose, can be depicted like in Fig. 1.1, but this linear structure is not very stable in watery solution. Instead, in a watery (or aqueous) solution the reaction in Fig. 1.2 is favored where an ether is formed, forming the characteristic ring structure. In these watery solutions, more than 95% of glucose molecules will adopt a ring structure. Glucose has two isoforms, L and D, but only the latter is found in nature. After forming a ring structure, there are also two isoforms, alpha and beta. The configuration depends on the orientation of the groups when they react during the closure of the ring. Like any ring structure, the glucose ring experiences torsional strain. In addition, the abundance of polar groups that repel each other exacerbates this problem. As a result, it will adopt one of two possible chair structures (see Fig. 1.3). The left one (C1 pointing down) is slightly more stable, making it the most stable glucose isomer in solution.
Fig. 1.1 The chemical structures of both glucose (left) and fructose (right). Note these molecules are structural isomers, they have the same atoms and differ only in the order of the atoms. Both molecules can exist in a linear form (top), but in watery solutions >95% will exist as a ring structure (bottom). Retrieved from Wikimedia Commons (TL, TR, BL, BR), Public Domain.#
Fig. 1.2 In watery solutions, the conversion of the linear structure of glucose (left) into the ring structure (right) is favored. This conversion happens through an etherification reaction (middle). Adapted from Wikimedia Commons (left, right), Public Domain.#
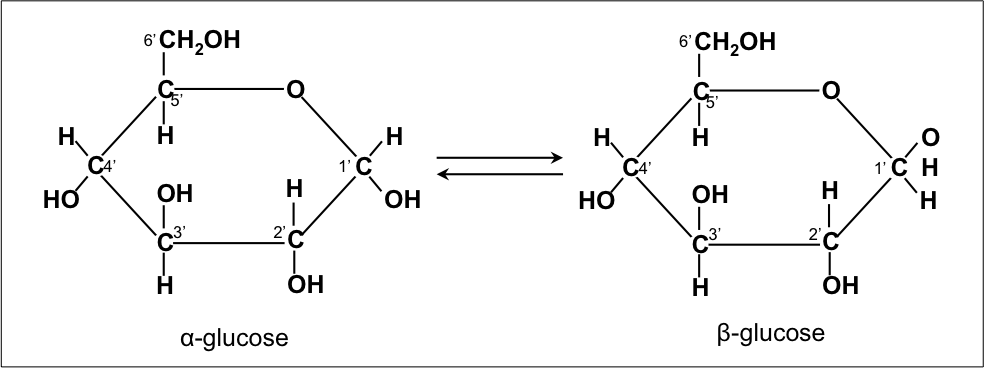
Fig. 1.3 The two anomers of glucose in ring conformation. Notice the OH group of C1 is pointing either downwards (left) or upwards (right). Of the two, α-glucose (left) is the most stable, making it the most common isomer of glucose in watery solutions. Retrieved from Wikimedia Commons, Public Domain.#
As you can see from the structures, monosaccharides contain many hydroxyl groups and therefore many chiral centers. This means there is a lot of potential for isomerization. Structural isomers have the same number of atoms, but in a different configuration. Stereoisomers have the same configuration but a different spatial arrangement. Enantiomers are mirror images, these are common in organic chemistry but not in biochemistry. Diastereoisomers have some chiral centers that are mirror images while other centers are identical. Among these you have two subtypes, epimers and anomers. In epimers exactly one of the chiral centers is mirrored while the others are not. In anomers the asymmetry is formed due to a ring closing. In addition to the diversity provided by this isomerism, monosaccharides can also be chemically modified, see Fig. 1.4. As you can see, these modifications range from smaller changes, like the addition of a methyl group in fucose, to larger modifications like the addition of an acetyl through an amine bond to form acetylglucosamine. There can even be multiple additions, as can be seen in sialic acid. These are of course but three examples of the many different modifications that exist in nature. This immense diversity, in chemical structures, isomers and modifications makes the study of carbohydrates rather complex.
Fig. 1.4 Different modifications of glucose. The original glucose structure is given in black while the modifications are shown in magenta.#
1.2.2. Polysaccharides#
Two monosaccharides can react through a condensation reaction to form a disaccharide. In fact, the sugar we buy in grocery stores is actually the disaccharides sucrose. Sucrose is composed of two different monosaccharides, a glucose and a fructose molecule. Many of the common sugars in our diets are actually disaccharides, other examples include lactose and maltose. These disaccharides are all produced in the same way; the main difference is their constituent monosaccharides. For example, there is a glucose molecule in lactose like there is in sucrose, but in this case the second monosaccharide is galactose. There are different configurations in which two saccharides can bind (see Fig. 1.5).
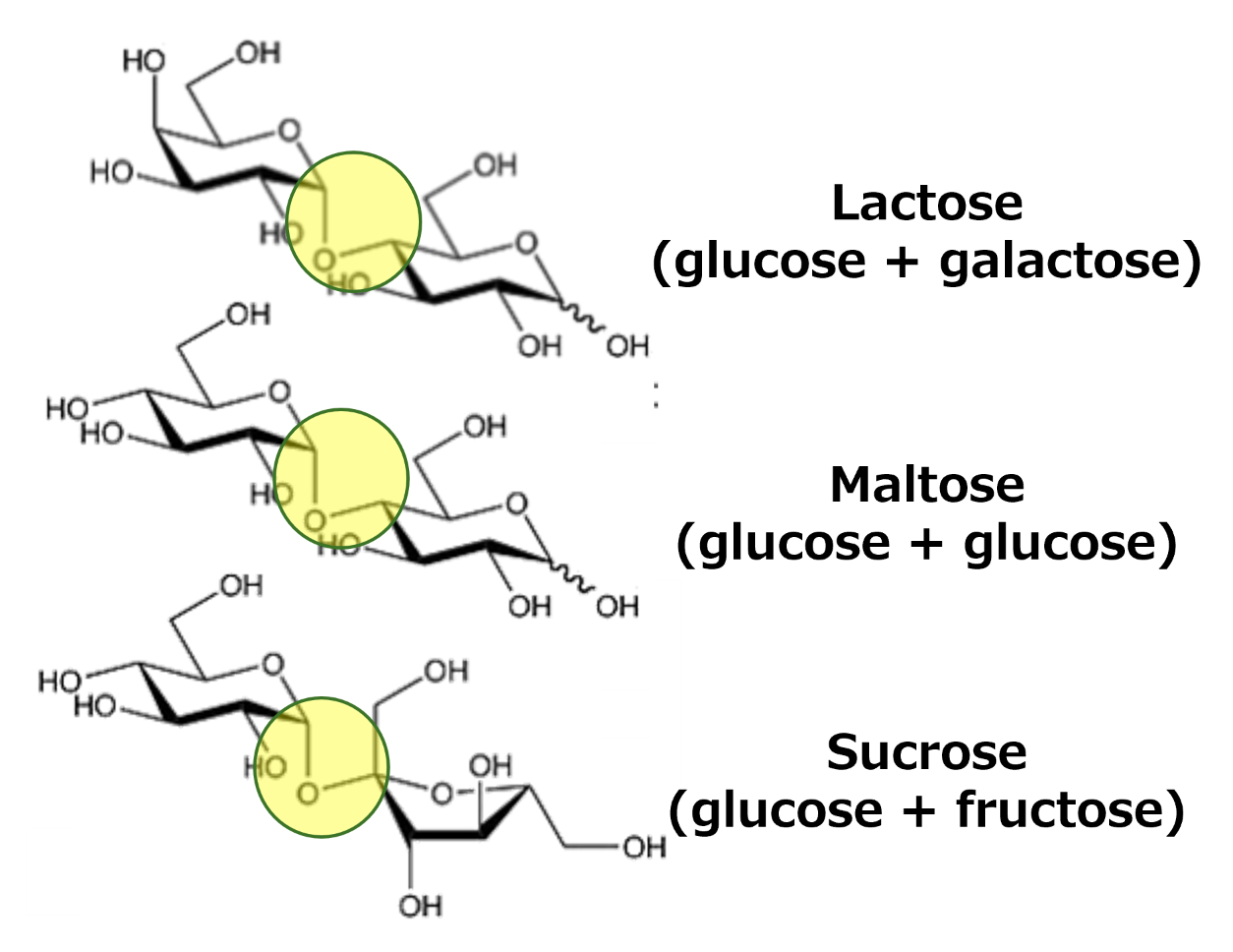
Fig. 1.5 The three disaccharides which are most important for our diets. As can be seen, they all have the same basic structure of two saccharides linked by an ether bond. The difference between the different disaccharides is the combination of saccharides. Retrieved from Wikimedia Commons, Public Domain.#
Maltose is a combination of two glucose molecules, but while this disaccharide is an important part of our diets, it tends to not be as well-known. This is because unlike the other two, we tend to consume maltose as part of a larger structure, the polysaccharide starch. Maltose is one of starch’s breakdown products during digestion. When more than two sugars are linked, we call them polysaccharides. These can be either linear (also known as unbranched) or branched. Polysaccharides have two main functions: providing structure and storing energy. Structural components are molecules like cellulose or chitin, storage includes molecules like starch or glycogen.
We will first take a closer look at the structural polysaccharides. If we look more closely at cellulose, we can see it is a linear polysaccharide composed entirely of glucose. These glucose molecules are linked together with a beta-1,4-glycosidic bond. Chitin has the same basic structure, but is composed of a different, modified sugar. Between different strands of cellulose there are extensive hydrogen bonds because of the many hydroxyl groups. This abundance of hydrogen bonds makes the connection between different cellulose strands very strong. It is precisely this strength which makes them suitable as structural components. The same principle applies to chitin, though the details are slightly different because of the different sugars. In both cases, the extensive hydrogen bonds allow the strands to form macromolecular structures like plates or fibers.
The structure of energy storage polysaccharides, such as starch and glycogen, is very different. These polysaccharides have an alpha-1,4-bond and an additional 6-bond. This additional bond allows these polysaccharides to be branched, forming large complex structures. The alpha-1,4-bonds are kinked which allows them to assemble into a helical structure. Like their structural counterparts, these helical structures are stabilized by extensive internal hydrogen bonds. The branched, helical nature of the starch and glycogen allow them to be very compacted and as a result they form granules that are easily visible with a microscope.
1.2.3. Glycoconjugates#
As we have seen, sugars are very versatile molecules that can react with one another to form polymers with a variety of properties. However, sugars can also react with other types of molecules, such as proteins and lipids. These modifications allow for a staggering number of options and properties. For example, while glycoproteins are found in many functions, they are very common on the outside of the cell. Here, these outside proteins are glycosylated, and sugar molecules are added in a specific order and pattern. Because of this specific pattern, these glycoproteins are crucial for cell-cell recognition as well as immune recognition. There are also multiple types of glycolipids, especially a class called glycosphingolipids is physiologically important. Here too, the addition of sugars plays a role in cell interaction and differentiation.
1.3. Lipids#
1.3.1. Types of lipids#
Lipids, another major class of biomolecules, are very important in three different ways. First, they form the bulk of the membrane, the outer layer of the cell. Second, they can also act as energy storage. Finally, they can be released and have a signaling function similar to hormones. Each of these functions is carried out by a different type of lipid (see Fig. 1.6). For energy storage, the main lipid type are the triacylglycerols. These molecules are composed of a glycerol that has three fatty acids attached (esterified). As you can imagine, having three fatty acids makes this molecule very hydrophobic. Because of this hydrophobicity they exist intracellularly as fat droplets, which are predominantly numerous in so-called fat cells, the cells that constitute our fat tissues. Phospholipids are the lipid type that make up the bulk of membranes. Their structure is similar to triacylglycerol, but with one major difference: instead of three fatty acids, phospholipids only have two. The second hydroxyl group of glycerol is esterified to a phosphate or a phosphate connected to another small molecule (choline, serine, inositol, ethanolamine), the so-called headgroup. These headgroups are hydrophilic, making phospholipid amphipathic. There are various possible headgroups of various complexity and each confers different properties. The lysoform of phosphatidic acid (a phospholipid containing only phosphate as its headgroup) form function as signaling lipids. Lyso means that one of the fatty acids is removed. Removing a fatty acid makes the molecule considerably more soluble, allowing it to be transported in the blood.
Fig. 1.6 The main type of lipid molecules. Retrieved from Wikimedia Commons, Public Domain.#
1.3.2. The membrane#
Because of the amphipathic nature of phospholipids, they are very suitable to membrane formation as their dual properties allows them to self-assemble into bilayers (see Fig. 1.7). Bilayers have a particular organization, where the hydrophobic fatty acids face each other while the hydrophilic headgroups face the surrounding aqueous solution. While they are the main component, membranes are not pure phospholipid bilayers. There are many other types of molecules inside membranes, and they all have important functions. For example, while they have a bad reputation, cholesterols are a vital part of a healthy membrane. These small hydrophobic molecules are embedded in the membrane and sit among the fatty acids. Because they take up space, this reduces how tightly packed the membrane is, and so they help keep the membrane fluid. There are also another class of lipids, the sphingolipids. Sphingolipids are considerably more rigid compared to phospholipids and have a stabilizing role in the membrane. Furthermore, sphingolipids are the only lipids that can be glycosylated (glycosphingolipids).
In our cells, as well as those of most organisms, the membrane is asymmetric. This can be shown by separating the inner and outer leaflets. If you measure the composition of the two leaflets you can see that some types of phospholipids are preferentially located in one leaflet. For example, phosphatidylethanolamine is located predominantly in the inner leaflet (facing the cytoplasm) while phosphatidylcholine is predominantly located in the outer leaflet. Phosphatidylserine is even exclusively located in the inner leaflet. These asymmetries are no accident and are tightly controlled. For example, if there is any phosphatidylserine present in the outer leaflet, it means the cell may be going into apoptosis.
In our membrane, all the lipids have an ester link between the fatty acids and the headgroup. This is not the case for all organisms, and in fact this is one of the reasons archaea are different from bacteria and eukaryotes. In archaea there are lipids that have an ether link between the fatty acids and headgroup. This type of lipid bond is found almost exclusively in archaea, though there are also remnants of this even in our own bodies. The platelet activating factor is a lipid signaling factor that contains an ether link. This is most likely a remnant of evolution, it is likely the only example of an ether linked lipid in vertebrates.
Membranes are not just present at the outer edge of the cell, but there are also numerous intracellular membranes. These membranes serve to create subdivisions inside the cell. For example, there is a membrane around the nucleus, the nuclear envelope. Other examples are organelles such as the endoplasmic reticulum and Golgi system which are largely consistent of membranes. There are of course also the mitochondria, which has its own membrane. Finally, there are also smaller granules used for transport and secretion. All of these intracellular membranes can be divided into two types. While most consist of a single membrane, there are two organelles, the mitochondrion and chloroplasts, that contain a double membrane. This means there are two separate bilayers, and this has an important physiological function that will be discussed in a later chapter. All the other membranes found in eukaryotic cells consist of only a single membrane. However, that does not mean that all these membranes are the same. There are large compositional differences between each of the intracellular membranes.
How do these membranes self-assemble? If you simply put some phospholipids into an aqueous solution, it will form a small droplet called a micelles (see Fig. 1.7). In these micelles the hydrophobic fatty acids are facing inward, creating a local hydrophobic environment, while the hydrophilic headgroups are facing the surrounding water molecules. Micelles are spherical, which means that each lipid molecule has to assume a conical shape, which is not energetically favorable. What this means is that if you start adding more lipids, once a critical mass is reached, they will spontaneously assemble into a bilayer, a sheet. If these bilayers get large enough, they will take the shape of a vesicle, a spherical bilayer surrounding an aqueous cavity. In research vehicles are frequently used as the basis for a synthetic cell.
Fig. 1.7 A schematic overview of the different types of structures which phospholipids can form in aqueous solutions. At low concentrations of phospholipids, they will first form a micelle (right). As the number of phospholipids increases, they will spontaneously form a bilayer (bottom). Once these become large enough, they will form a spherical structure enclosing an aqueous solution, a vesicle. Adapted from Wikimedia Commons, Public Domain.#
Fatty acids are very long chains, generally between 14 and 20 carbon long, depending on the organism. Due to hydrophobic interactions, this long length reduces the freedom of movement considerably, which has important consequences for the fluidity of the membrane. The length of the carbon chain, the presence or absence of double bonds and the amount of cholesterol in the membrane all determine how much individual phospholipids can move within the plane of the membrane. This ability to move gives the membrane a degree of what is called fluidity (see Fig. 1.7). Fluidity is very important for the membrane, but highly dependent on factors like lipid composition and of course the temperature. If you take fluid membrane and cool it down below its transition temperature, the lipids will reorganize into a more rigid crystalline like organization and this inhibits movement. This transition temperature can be equated to a melting temperature. Butter provides an apt analogy, when we grab a stick of butter from the fridge it is solid and rigid (akin to the crystalline state). Once it has sat on the countertop for a few minutes and has warmed to room temperature, we can notice its fluidity has changed. The butter is still solid but has become much softer and pliable (akin to the ripple phase). Only when placed into a hot pan does it fully melt (akin to the fluid phase, though more extreme). Membrane fluidity is hugely important for cells, because a rigid membrane does not function well, nor does a fully fluid membrane. The composition of the lipid is very important for where this transition temperature is. Lipids with longer fatty acids have a lower transition temperature. Furthermore, the degree of saturation is also important, the more unsaturated the lower the transition temperature (see Fig. 1.8).
Fluidity of the membrane allows for movement of lipid molecules. There are two types of movement a lipid molecule can undertake. Lateral diffusion, where a lipid molecule moves to a different spot in the same leaflet, is a fast process. The other type of movement, transverse diffusion, is very slow. During transverse diffusion a lipid flips from the outer leaflet to the inner leaflet, or it flops from the inner leaflet to the outer leaflet. However, to do this the hydrophilic headgroup has to pass through the hydrophobic layer of fatty acids. This is so energetically unfavorable that it almost doesn’t happen spontaneously. Yet, as discussed previously, there is an asymmetry between the inner and outer leaflets, which means that transverse diffusion has to happen somehow. The way cells manage this is through the use of enzymes. These enzymes can use ATP to move a lipid to the inner leaflet (flippases) or the outer leaflet (floppases).
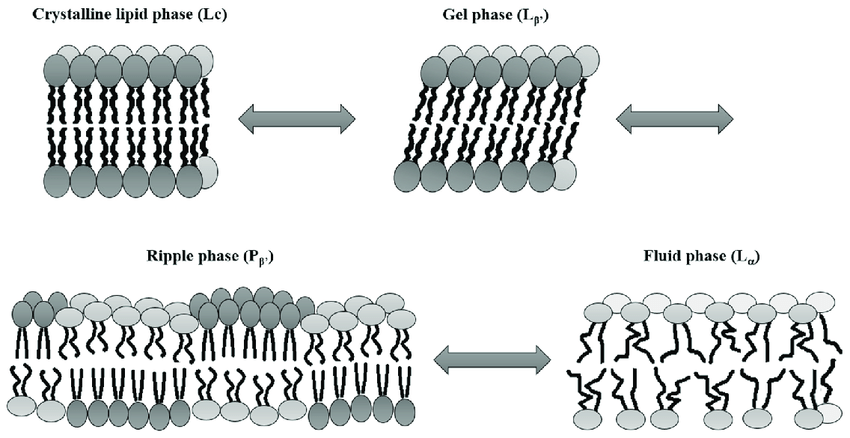
Fig. 1.8 A schematic drawing showing the different phases a lipid membrane can have, from the most ordered and solid state (top left) to the most liquid disordered state (bottom right). The fluidity of a membrane is affected by many parameters, such as the composition of the membrane and the temperature. Image retrieved from [1], licensed under CC-BY.#
Fig. 1.9 A cartoon showing the effect of adding unsaturated phospholipids (blue) to a membrane. Because of the unsaturated double bound, these phospholipids have a kink in their acyl chains. This means they take up more space compared to saturated phospholipids and thus disrupt the tight packing of the lipid membrane. Retrieved from Wikimedia Commons, Public Domain.#
Membranes have several uses. One is of course compartmentalization by acting as a physical barrier. In this way the cell is able to create different conditions (e.g. pH, charge or metabolite concentrations) in different areas. But membranes aren’t equal opportunity barriers. While generally impermeable to larger molecules like proteins and DNA, smaller molecules such as water and particular ions can sometimes diffuse across membranes depending on their properties. By including active transport enzymes membranes, energized by ATP hydrolysis, can be used to create a gradient of molecules and ions. Gradients are a source of potential energy that can be used for many physiological processes. Finally, for secretion, uptake and cell division the ability of membranes to bud and fuse (exo- and endocytosis) is important. In many processes the properties of the membrane are important, but the actual work and decision-making often falls to proteins. For example, gradients can only be established because there is a protein facilitating the active, directional transport of the small molecule. There are several types of membrane proteins (see Fig. 1.10). Peripheral membrane proteins don’t fully enter the membrane, but instead skim the surface. Integral membrane proteins are fully inserted into the membrane. If an integral membrane protein goes through both leaflets they are called transmembrane proteins. Many transmembrane proteins facilitate transport across the membrane, often featuring a protein channel.
Fig. 1.10 A schematic overview of a membrane showing the most common molecules (phospholipids, glycolipids and cholesterol) as well as the main types of proteins found at the membrane. Adapted from Wikimedia Commons, licensed under CC-BY-SA 3.0.#
J. Startek, B. Boonen, Talavera K., and V. Meseguer. Trp channels as sensors of chemically-induced changes in cell membrane mechanical properties. International Journal of Molecular Sciences, 20(2):371, 2019. doi:10.3390/ijms20020371.